Potential Risk of Choline Alfoscerate on Isoflurane-Induced Toxicity in Primary Human Astrocytes
Article information
Abstract
Objective
Isoflurane, a widely used common inhalational anesthetic agent, can induce brain toxicity. The challenge lies in protecting neurologically compromised patients from neurotoxic anesthetics. Choline alfoscerate (L-α-Glycerophosphorylcholine, α-GPC) is recognized for its neuroprotective properties against oxidative stress and inflammation, but its optimal therapeutic window and indications are still under investigation. This study explores the impact of α-GPC on human astrocytes, the most abundant cells in the brain that protect against oxidative stress, under isoflurane exposure.
Methods
This study was designed to examine changes in factors related to isoflurane-induced toxicity following α-GPC administration. Primary human astrocytes were pretreated with varying doses of α-GPC (ranging from 0.1 to 10.0 μM) for 24 hours prior to 2.5% isoflurane exposure. In vitro analysis of cell morphology, water-soluble tetrazolium salt-1 assay, quantitative real-time polymerase chain reaction, proteome profiler array, and transcriptome sequencing were conducted.
Results
A significant morphological damage to human astrocytes was observed in the group that had been pretreated with 10.0 mM of α-GPC and exposed to 2.5% isoflurane. A decrease in cell viability was identified in the group pretreated with 10.0 μM of α-GPC and exposed to 2.5% isoflurane compared to the group exposed only to 2.5% isoflurane. Quantitative real-time polymerase chain reaction revealed that mRNA expression of heme-oxygenase 1 and hypoxia-inducible factor-1α, which were reduced by isoflurane, was further suppressed by 10.0 μM α-GPC pretreatment. The proteome profiler array demonstrated that α-GPC pretreatment influenced a variety of factors associated with apoptosis induced by oxidative stress. Additionally, transcriptome sequencing identified pathways significantly related to changes in isoflurane-induced toxicity caused by α-GPC pretreatment.
Conclusion
The findings suggest that α-GPC pretreatment could potentially enhance the vulnerability of primary human astrocytes to isoflurane-induced toxicity by diminishing the expression of antioxidant factors, potentially leading to amplified cell damage.
INTRODUCTION
As the population continues to age significantly, an increasing number of older individuals are undergoing neurosurgical procedures managed under general anesthesia. Advanced age has been identified as a risk factor for postoperative cognitive dysfunction, a condition where cognitive deterioration below the baseline occurs the day after surgery [12,29]. This cognitive dysfunction presents itself as deficits in learning, memory, language, attention, and executive functions. Although the exact cause of postoperative cognitive dysfunction remains uncertain, current research primarily focuses on anestheticinduced neuroinflammation and oxidative stress, which could lead to cell damage and death [38]. Several in vivo studies suggest that the inhalation of anesthetic agents could exacerbate neuronal cell death through degradation of the cholinergic system involved in consciousness, learning, and memory [28,30]. Isoflurane, one of the most commonly used inhalational anesthetic agents, has also been associated with neuroinflammation and neuronal apoptosis [8]. Therefore, protecting the brain against these neurotoxic inhalational anesthetics is a critical challenge that must be addressed when treating neurologically-impaired individuals, including the elderly.
Choline alfoscerate, or L-alpha-glycerylphosphorylcholine (α-GPC), is a globally utilized cholinergic drug that contributes to cognitive function maintenance. Upon oral administration, α-GPC is metabolically converted into phosphatidylcholine, the active form of choline, which supplements acetylcholine in brains deficient in choline. The neuroprotective properties of α-GPC have been observed to aid cognitive recovery in patients dealing with dementia, acute stroke, and transient ischemic attacks [2,10]. Moreover, it is also reported to prevent ischemia-induced oxidative stress and inflammation by preserving mitochondrial respiratory function [32]. The above evidence suggests that α-GPC may impact brain cells from anesthetic-induced toxicity. However, recent studies have indirectly suggested that this drug might have specific indications or dosages, emphasizing the importance of correct dosage administration for safety purposes [19,37]. This prompted us to conduct an in vitro experiment to investigate the effects of pretreatment with α-GPC prior to isoflurane exposure. This experiment mirrors the administration of isoflurane for general anesthesia in patients who routinely take α-GPC.
The target cells of the current study were astrocytes, which remain the most abundant cell type in the brain. The function of astrocytes as protectors against oxidative stress is increasingly emphasized, along with their involvement in cognitive function [11,18]. Previous studies on α-GPC were predominately focused on neurons, specifically on its neuroprotective effects in disease models, such as Alzheimer’s disease [4,5] or post-seizure cognitive impairment [20]. Nevertheless, the effects of α-GPC on human astrocytes remain to be determined. Therefore, the primary objective of this study was to investigate the effects of α-GPC pretreatment on human astrocytes exposed to isoflurane using primary human astrocytes. We hypothesized that heme oxygenase-1 (HO-1), a stress-inducible enzyme that mediates cellular redox homeostasis with antioxidant effects to protect the cells, may have a role in mediating α-GPC effects. Hypoxia-inducible factor-1α (HIF-1α), which is related to isoflurane-induced toxicity, is also presumed to be involved. To this end, our secondary goal was to investigate whether α-GPC pretreatment modifies the expression of these enzymes and is reflected at the phosphorylation level of intracellular signaling components.
MATERIALS AND METHODS
These cells were commercially available and had no personally identifiable information associated with them. Consequently, IRB approval was exempted.
Primary human astrocytes culture
Primary human astrocytes from normal brain tissue were purchased from Gibco (Catalog No. N7805-100; Thermo Fisher Scientific, Waltham, MA, USA). Stock was immediately placed in a liquid nitrogen tank upon receipt. Prior to thawing and seeding the cells, the culture flasks were pre-coated with Geltrex matrix (Catalog No. A1413201; Thermo Fisher Scientific) which was diluted in Dulbecco’s Modified Eagle Media (Catalog No. 10569010; Thermo Fisher Scientific) for 1 hour at 37°C. Next, the coating solution was removed, and the flask surface was washed with Dulbecco’s phosphate-buffered saline (DPBS) including calcium and magnesium. The cells were incubated in complete media which contained 1% N2 supplement and 10% fetal bovine serum, in a humidified atmosphere of 5% CO2 at 37°C. Accutase (Catalog No. A1110501; Thermo Fisher Scientific) was used to detach the cells and all plastic wares were pre-wet with complete medium before adding the astrocytes containing medium.
Immunocytochemistry and cell imaging
For immunocytochemical staining, primary human astrocytes and human fibroblasts (CCD-986sk; ATCC, Manassas, VA, USA) were seeded on sterile glass cover slips and incubated for 24 hours in a six-well cell culture plate. Incubated cells were rinsed with DPBS, fixed with 4% (w/v) paraformaldehyde for 15 minutes at room temperature, permeabilized with 0.1% (v/v) Triton X-100 (Catalog No. T8787; Sigma-Aldrich, St. Louis, MO, USA) for 10 minutes. After rinsing thoroughly in DPBS, 3% (w/v) bovine serum albumin (Catalog No. 821001; Sigma-Aldrich) was applied to each chamber for 1 hour at room temperature for blocking. And then, cells were incubated at 4°C overnight with primary antibodies against glial fibrillary acidic protein (GFAP; Catalog No. ab4648; Abcam, Cambridge, UK), vimentin (Catalog No. ab8978; Abcam), cytokeratin (Catalog No. M351529-2; Agilent Technologies, Santa Clara, CA, USA) and synaptophysin (Catalog No. PA5-27286; Invitrogen, Carlsbad, CA, USA) diluted in 1% (w/v) BSA/0.1% (v/v) Triton X-100/DPBS (1 : 50–1 : 200). On the next day, cells were rinsed three times in DPBS and then incubated with the Alexa Fluor 488-conjugated secondary antibody (Catalog No. A-11001; Invitrogen) for 1 hour at room temperature. After being rinsed thoroughly in buffer, mounted with 4',6-diamidino-2-phenylindole mounting medium (Catalog No. H-1200-10; Vector Laboratories, Newark, CA, USA), sealed with cover slips. Images were taken with EVOS FL Auto 2 Cell Imaging System (Thermo Fisher Scientific).
α-GPC and isoflurane treatment
Culture plates of primary human astrocytes were placed in a humidified air-tight modular chamber (Catalog No. 27310; STEM CELL Technologies, Vancouver, Canada) and incubated at 37°C with inlet and outlet ports. The inflow port was connected to a vaporizer to deliver isoflurane gas with premixed carrier gas (21% O2, 5% CO2, and balanced nitrogen) or carrier gas alone at 2 L per minute. The outflow port was connected to a gas analyzer monitor (Catalog No. B105M; GE Healthcare, Waukesha, WI, USA) that measured isoflurane concentration. Exposure to isoflurane (2.5%, 4.0%, and 5.5%) was maintained for 24 hours and the control group was exposed to carrier gas in a parallel chamber condition. To assess the effect of α-GPC pretreatment, primary human astrocytes were pretreated with 0.1 µM, 1.0 µM and 10.0 µM α-GPC (Catalog No. G5291; Sigma Aldrich) for 24 hours before isoflurane exposure. To assess the effect of α-GPC pretreatment, primary human astrocytes were pretreated with 0.1 µM, 1.0 µM, and 10.0 µM α-GPC (Catalog No. G5291; Sigma Aldrich) for 24 hours before isoflurane exposure for preconditioning. Consequently, both the group treated only with α-GPC and the group co-treated with α-GPC and 2.5% isoflurane underwent a total of 48 hours α-GPC treatment.
Previous studies investigating the cellular toxicity of isoflurane have reported effects across varying exposure durations. In one study focused on the mechanisms of isoflurane neurotoxicity, neurons were exposed to 2.4% isof lurane for 12 hours [7], while another study investigating toxicity mechanisms in astrocytes exposed them to 3% isoflurane for 24 hours [25]. These experimental designs, similar to ours, were intended to induce cytotoxicity. Therefore, we deemed exposure durations of 24 hours to 2.5% isoflurane, which are longer than traditional surgical times, as necessary and acceptable for in vitro experiments.
Cell viability assay
Cell viability was assessed in more than triplicates per group using a colorimetric assay by evaluating the metabolic conversion of a water-soluble tetrazolium salt (WST-1; Catalog No. EZ-3000; DoGenBio Co., Ltd., Seoul, Korea). The viability was measured at various conditions in primary human astrocytes and assay were performed by adding WST-1 directly to the culture wells and incubating for 60 minutes at 37°C. The absorbance was measured at a wavelength of 450 nm.
Quantitative real-time polymerase chain reaction
Total RNA was extracted using the AccuPrep Universal RNA extraction kit (Catalog No. K-3140; Bioneer, Daejeon, Korea). Total 400 ng of RNA was then subjected to reverse transcription using AccuPower RT PreMix (Bioneer) according to the manufacturer’s instructions. The genes to be analyzed for expression level were survivin, HO-1, and HIF-1α. Quantitative real-time polymerase chain reaction (qRT-PCR) was performed in a final volume of 20 µL containing cDNA and each primer set using the Quant Studio Real-time PCR Software (Thermo Fisher Scientific) with a thermos cycler profile of 50°C for 2 minutes, 95°C for 10 minutes, and followed by 40 cycles of 95°C for 15 seconds, and 60°C for 1 minute. Each sample was evaluated in triplicate. Primer for GAPDH (forward 5’- AAT CCC ATC ACC ATC TTC CA-3’ and reverse 5’- TGG ACT CCA CGA CGT ACT CA-3’) was used as the internal control for normalizing relative mRNA expression on the basis of different cycle threshold (Ct) values. The PCR primer nucleotide sequences were as follows : HO-1, forward 5’-CTC AAA CCT CCA AAA GCC-3’, reverse 5’-GGG TTG GGG TGG TTT TTG A-3’; HIF-1α, forward 5’-CTG ACC CTG CAC TCA ATC AAG-3’, reverse 5’-TGG GAC TAT TAG GCT CAG GTG-3’; survivin, forward 5’-GGC CCA GTG TTT CTT CTG CTT-3’, reverse 5’-GGC CCA GTG TTT CTT CTG CTT-3’.
Proteome profiler array
Total protein was extracted with RIPA Lysis buffer (Catalog No. 89900; Thermo Fisher Scientific) from the control and experimental groups of primary human astrocytes. After protein quantification, 120 µg of each protein lysate were incubated overnight at 4°C according to the protocol of Proteome Profiler Human Phospho-Kinase array kit (Catalog No. ARY003C; R&D Systems, Minneapolis, MN, USA). The positive signals visible on the developed X-ray film can be identified by placing the transparency overlay on the array image and aligning it with the pairs of reference dots at the edge of each membrane. Expression densities of each dot were analyzed using a transmission mode scanner and ImageJ ver. 1.53t (National Institutes of Health, Bethesda, MD, USA) imaging analysis software.
Transcriptome sequencing
The extracted total RNA samples stored at -70°C were sent to the Next Generation Sequencing Service division of Macrogen Inc. (Seoul, Korea). The library was prepared with SMART-Seq™ v4 Ultra™ Low Input RNA Kit and TruSeq RNA Sample Prep Kit v2 following SMART-Seq™ v4 Ultra™ Low Input RNA library protocol. Sequencing was performed on the Illumina HiSeq platform with 101-bp paired-end reads. The preprocessed reads were mapped to the reference genome using the HISAT2 program, and transcript assembly was performed using the StringTie program. Expression profiles were extracted as FPKM (fragments per kilobase of transcript per million mapped reads)/RPKM (reads per kilobase of transcript per million mapped reads) values and TPM (transcripts per million) values considering read count, transcript length, and depth of coverage obtained through transcript quantification of each sample. In cases where known gene information was available, functional annotation and gene-set enrichment analysis based on the Kyoto Encyclopedia of Genes and Genomes (KEGG) database (http://www.genome.jp/kegg/) for differentially expressed genes were conducted [7,16,17].
Statistical analysis
Data were analyzed with GraphPad Prism 10.0 software (GraphPad Software, San Diego, CA, USA) and presented as the means±standard deviation. Comparison among groups was done using an unpaired two-tailed t-test with Welch’s correction for normally distributed data and a Mann-Whitney test for nonparametric data. Shapiro-Wilk tests were used to determine the normality of the data. p-value of <0.05 was regarded as statistically significant and was presented as p<0.05 (*), p<0.01 (**), p<0.001 (***), and p<0.0001 (****).
RESULTS
Verification of primary human astrocytes
As this study was intended to reach in cases of adult patients, including the elderly, mature astrocytes were targeted. The maturity and propriety of primary human astrocytes were confirmed through immunocytochemistry by assessing the expression of GFAP, vimentin, synaptophysin, and cytokeratin (Fig. 1A). For comparative purposes, human fibroblast cells were also cultured and stained. The presence and proportion of astrocytes in the cultured cells were determined using GFAP, a widely accepted marker for astrocytes. Positive GFAP staining was identified in over 98% of the cells. Vimentin, a specific marker for fibroblasts known to serve as an early marker of glial differentiation and expressed in glial precursors well before the onset of GFAP expression, was used to identify immature astrocytes [34]. Compared to the strong positive staining results on human fibroblasts, the cultured primary human astrocytes exhibited negative staining with vimentin. Synaptophysin and cytokeratin serve as markers for neuronal and epithelial cells, respectively. The astrocytes and fibroblasts did not exhibit synaptophysin or cytokeratin staining. According to the findings, the cultured primary human astrocytes were considered mature astrocytes, and it was confirmed that the primary human astrocytes being cultured were suitable for conducting this study.

A : Immunocytochemistry images of cultured primary human astrocytes (×100 magnification; green : Alexa Fluor 488-conjugated secondary antibody; blue : 4',6-diamidino-2-phenylindole). Human fibroblasts were used as a positive control for vimentin and a negative control for GFAP. The results indicate that the cultures were enriched with mature astrocytes. B : Morphological alteration of primary human astrocytes by isoflurane exposure in a dose-dependent manner (×100 magnification). The characteristics of morphological damage are manifested through cell shrinkage and diminished intercellular adherence as observed under a light microscope. The numbers depicted in the image denote the concentration of isoflurane. C : Schematic diagram of experimental protocol designed to evaluate the impact of α-GPC on isoflurane-induced toxicity. GFAP : glial fibrillary acidic protein, α-GPC : L-α-Glycerophosphorylcholine, WST : water-soluble tetrazolium salt, qRT-PCR : quantitative real-time polymerase chain reaction.
Determination of experimental doses of α-GPC and isoflurane
To determine the appropriate experimental doses of α-GPC and isoflurane, we initially conducted a dose-dependent morphological analysis of primary human astrocytes in the presence of isoflurane. In clinical practice, isoflurane concentrations of 2.0–4.0% are commonly used for induction during inhalation anesthesia, while concentrations of 0.5–2.0% are preferred for maintenance [13]. However, the settings for conducting in vitro experiments may differ from those in clinical settings. Previous in vitro studies investigating isoflurane-induced cytotoxicity on primary astrocytes were conducted by exposing the astrocytes to 3.0–3.5% isof lurane for 9–24 hours [14,25]. Accordingly, we selected an isoflurane concentration of 2.5% to establish experimental conditions for isoflurane-induced toxicity, and 4.0% and 5.5% as positive control concentrations. Under a light microscope, features indicative of cell damage, such as cell shrinkage and reduced adherence to neighboring cells, were observed at all tested isoflurane concentrations, beginning at 2.5% isoflurane (Fig. 1B).
In this study, we conducted experiments with 0.1 µM, 1.0 µM, and 10.0 µM of α-GPC as the preferred experimental concentrations. Recent in vitro studies examined α-GPC on neuronally differentiated SH-SY5Y cells using 0.1 mM, 1 mM, 10 mM, and 50 mM of α-GPC with pretretment of 24 hours and additional treatment of 24 hours with β‐amyloid treatment, similar to our experimental design [5]. Another study examined the effect of α-GPC on β‐amyloid-induced neurotoxicity by treating SH-SY5Y cells with 100 nM α-GPC 1 hour pretreatment and treating them with β‐amyloid treatment for 72 hours [4]. As concentration used in each study differs, we tried to determine the concentration range using a recent pharmacokinetic study on α-GPC. A recent pharmacokinetic study on choline alfoscerate, conducted using the currently prescribed choline alfoscerate capsule medication and a method of collecting human blood samples, found that when a typical adult dosage of 400 mg, taken three times a day (totaling 1200 mg), was administered orally, the mean area under the plasma concentration-time curve from time 0 to infinity was found to be 3.305±1.803 µg⋅h/mL in a bioequivalence study [27]. This corresponds to a value of 12.849±7.001 µM⋅h. Based on these results, we have decided to use α-GPC at a concentration of 10.0 µM and isoflurane at a concentration of 2.5% for the experiments. The design of subsequent experiments was based on these concentrations (Fig. 1C).
Effects of α-GPC and isoflurane on cell viability of primary human astrocytes
For further validation of the experimental settings, we conducted additional reference experiments in conjunction with the experimental plan outlined in Fig. 1C. These included cell viability experiments using α-GPC at concentrations of 0.1 µM and 1.0 µM, and isoflurane at concentrations of 4.0% and 5.5%. To verify the effect of α-GPC on the viability of primary human astrocytes, the astrocytes were treated with various concentrations of α-GPC (0.1, 1.0, and 10.0 µM) for 48 hours. The viability of these cells was subsequently analyzed using the water-soluble tetrazolium salt (WST)-1 assay (Fig. 2A, left). The results indicated that treatment with α-GPC did not significantly affect the viability of the astrocytes, as there were no significant differences in viability across the different α-GPC concentrations (control 1.07±0.03; α-GPC 0.1 µM, 1.07±0.07, not statistically significant [ns]; α-GPC 1.0 µM, 0.91±0.09, ns; α-GPC 10.0 µM, 1.04±0.04, ns). However, exposure to isoflurane resulted in a dose-dependent decrease in primary human astrocyte viability. Specifically, when the astrocytes were treated with isoflurane concentrations of 2.5%, 4.0%, and 5.5% for a period of 24 hours, there were significant reductions in cell viability by 14%, 32%, and 36% respectively, compared to the control group, as determined by the WST-1 assay (control, 1.00±0.03; isoflurane 2.5%, 0.86±0.07, p<0.01; isoflurane 4.0%, 0.68±0.03, p<0.0001; isoflurane 5.5%, 0.64±0.05, p<0.0001; Fig. 2A, right). The result reaffirmed that a 2.5% concentration of isoflurane can establish suitable experimental conditions that induce noticeable toxicity, such as decreased cell viability and morphological damage to the cells, without being so severe as to disrupt further studies, as depicted in Figs. 1B and 2B.
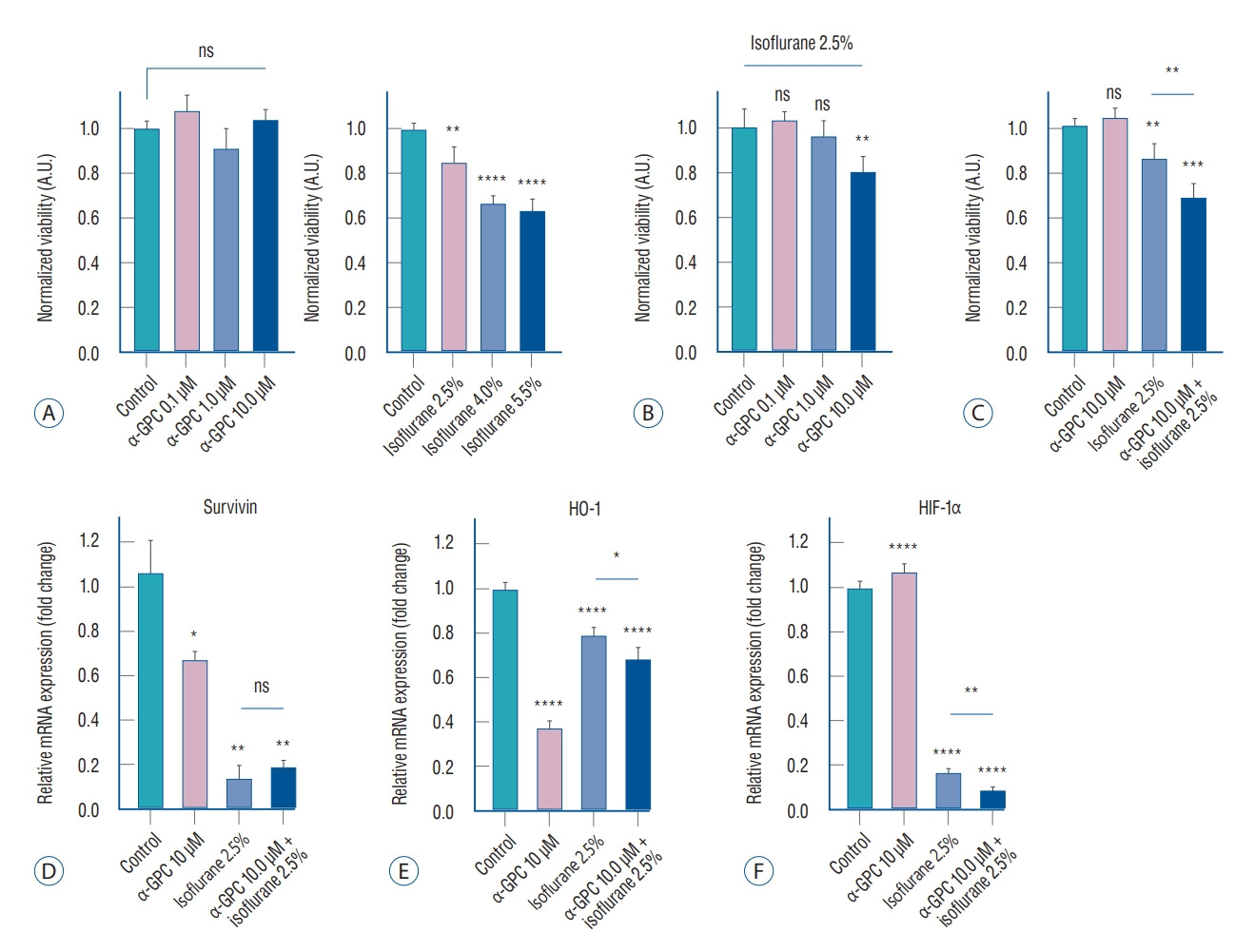
A : WST-1 cell viability assay results of primary human astrocytes subjected to α-GPC and isoflurane. There was no significant difference in cell viability at any dose of treatment with α-GPC for 48 hours compared to control (n=6 for each, left), but isoflurane exposure reduced cell viability in a dose-dependent manner (n=6 for each, right). B : The effect on cell viability by 0.1, 1.0, 10.0 μM of α-GPC pretreatment and 2.5% isoflurane exposure (n=6 for each). After 24 hours of exposure to 2.5% isoflurane, pretreatment with 10.0 μM of α-GPC significantly reduced cell viability by approximately 20% compared to the group not treated with α-GPC (n=6). C : Reanalyzed results considering the individual and combined effects of 10.0 μM of α-GPC and 2.5% isoflurane. Primary human astrocytes, when pretreated with 10.0 μM of α-GPC and subsequently exposed to 2.5% isoflurane, exhibited a 32% reduction in cell viability compared to the non-treated control group. This decrease was notably more substantial than the 14% reduction observed when cells were only treated with 2.5% isoflurane, compared to the non-treated control group. D : Analysis of survivin mRNA expression in each treatment group using quantitative real-time polymerase chain reaction (qRT-PCR). In comparison to the non-treated control group, all groups treated with either 10 μM of α-GPC, 2.5% isoflurane, or a combination of both, demonstrated a statistically significant decrease in survivin expression (n=3 for each). E : Analysis of heme oxygenase-1 (HO-1) mRNA expression in each treatment group using qRT-PCR (n=3 for each). The groups pre-treated with α-GPC exhibited a significant reduction in HO-1 mRNA expression when compared to the control group. F : Analysis of hypoxia-inducible factor-1α (HIF-1α) mRNA expression in each treatment group using qRT-PCR (n=3 for each). In all groups treated with 2.5% isoflurane, the HIF-1α expression significantly decreased by more than 80%. The results are presented as the means±standard deviations. *p<0.05, **p<0.01, ***p<0.001, ****p<0.0001. A.U. : arbitrary unit, ns : not statistically significant, α-GPC : L-α-Glycerophosphorylcholine, WST-1 : water-soluble tetrazolium salt.
To evaluate the effect of α-GPC pretreatment on the response of primary human astrocytes to isoflurane, astrocytes were pretreated with α-GPC at concentrations of 0.1, 1.0, and 10.0 µM for 24 hours before being exposed to 2.5% isoflurane for an additional 24 hours. The WST-1 assay revealed that pretreatment with 0.1 µM and 1.0 µM of α-GPC did not affect cell viability after 24 hours of exposure to 2.5% isoflurane (control, 1.00±0.08; α-GPC 0.1 µM + isoflurane 2.5%, 1.03±0.04, ns; α-GPC 1.0 µM + isoflurane 2.5%, 0.96±0.07, ns; Fig. 2B). However, pretreatment with 10.0 µM of α-GPC significantly reduced cell viability by approximately 20% compared to the non-α-GPC treated group following 2.5% isoflurane exposure (α-GPC 10.0 µM + isoflurane 2.5%, 0.80±0.07, p<0.01; Fig. 2B). Upon reanalyzing the results, it was discovered that cell viability decreased by 32% compared to the non-treated control, which was not treated with either α-GPC or isoflurane, when the cells were pretreated with α-GPC at a concentration of 10.0 µM and exposed to 2.5% isoflurane (control, 1.00±0.03; α-GPC 10.0 µM, 1.04±0.04, ns; isoflurane 2.5%, 0.86±0.07, p<0.01; α-GPC 10.0 µM + isoflurane 2.5%, 0.68±0.06, p<0.01; Fig. 2C). This decrease was significantly greater than the 14% reduction observed when cells were treated only with isof lurane at 2.5%, compared to the non-treated control (p<0.01; Fig. 2C).
Alterations in apoptosis- and oxidative stress-related cell signals induced by α-GPC and isoflurane
In primary human astrocytes, where isoflurane-induced toxicity was observed, it was found that the group pretreated with 10 µM of α-GPC exhibited a significant decrease in cell viability compared to the group not pretreated with α-GPC. To investigate the cell signaling mechanism underlying this phenomenon, the expression of ‘Survivin’, a representative inhibitor of apoptosis protein, was initially examined using qRT-PCR [31]. A statistically significant decrease in survivin expression was observed in all groups treated with 10 µM of α-GPC, 2.5% isoflurane, and both, compared to the control group (control, 1.06±0.14; α-GPC 10 µM, 0.67±0.04, p<0.05; isoflurane 2.5%, 0.14±0.06, p<0.01; α-GPC 10 µM + isoflurane 2.5%, 0.19±0.03, p<0.01; Fig. 2D).
For further validation and investigation of related cell signaling, experiments were also conducted on the expression of HO-1 and HIF-1α, which are thought to be one of the major cell signals associated with protecting cells from oxidative stress and attenuating oxidative stress-induced apoptosis [1,3,22,23]. The mRNA expression of HO-1 was significantly reduced in the groups pretreated with α-GPC compared to the control group (control, 1.00±0.03; α-GPC 10 µM, 0.37±0.04, p<0.0001; isoflurane 2.5%, 0.79±0.04, p<0.0001; α-GPC 10 µM + isoflurane 2.5%, 0.68±0.06, p<0.0001; Fig. 2E). The increase in HO-1 mRNA expression in the group treated only with 2.5% isoflurane, compared to the group treated only with 10 µM of α-GPC, is considered a compensatory increase in response to oxidative stress induced by isoflurane. However, a significant reduction was also observed between the ‘isoflurane 2.5%’ group and the ‘α-GPC 10 µM + isoflurane 2.5%’ group (p<0.05), as shown in Fig. 2E. These results can be interpreted as indicating that pretreatment with 10 µM of α-GPC reduces the HO-1 mRNA expression of primary human astrocytes, which may result in increased vulnerability to oxidative stress.
In the case of HIF-1α, there was no significant difference in HIF-1α expression between the group treated only with 10 µM of α-GPC and the control group. However, in all groups treated with 2.5% isoflurane, there was a significant decrease of more than 80% in HIF-1α expression (control, 1.00±0.03; α-GPC 10 µM, 1.07±0.04, ns; isoflurane 2.5%, 0.17±0.02, p<0.0001; α-GPC 10 µM + isof lurane 2.5%, 0.09±0.15, p<0.0001; Fig. 2F). Furthermore, a more statistically significant decrease was observed in the ‘α-GPC 10 µM + isoflurane 2.5%’ group compared to the ‘isoflurane 2.5%’ group (p<0.01; Fig. 2F). These results suggest that treatment with 10 µM of α-GPC under 2.5% isoflurane exposure may contribute to the suppression of HIF-1α expression in primary human astrocytes, and as a result, the astrocytes may become more susceptible to oxidative stress-induced apoptosis.
To further investigate changes in cell signaling associated with isoflurane-induced toxicity and the potential involvement of 10 µM α-GPC, beyond those related to oxidative stress-induced apoptosis, we conducted a phosphorylation assay and transcriptome sequencing analysis using next-generation sequencing. We utilized a membrane- and antibody-based Proteome Profiler Human Phospho-Kinase array to compare the levels of protein phosphorylation involved in the intracellular signaling pathway alteration induced by 2.5% isoflurane and pretreatment with 10.0 µM of α-GPC (Fig. 3A). Upon comparing the results between the ‘isoflurane 2.5%’ group and ‘α-GPC 10.0 µM + isoflurane 2.5%’ group, we observed differential expression of heat shock protein 60 (HSP60), signal transducer and activator of transcription3 (STAT3), 90 kDa ribosomal S6 kinase 1/2 (RSK1/2), p53, and epidermal growth factor receptor (EGFR) upon gross inspection. Pixel density signals from spots were averaged, and quantitative analysis indicated increased (EGFR, 1.46-fold) or decreased (HSP60, 0.63-fold; STAT3, 0.63-fold; RSK1/2, 0.73-fold; p53, 0.92-fold) phosphorylation of these proteins when the levels of the ‘α-GPC 10.0 µM + isoflurane 2.5%’ groups were compared to those of the ‘isoflurane 2.5%’ group.

A : Phosphorylation screening analysis using proteome profiler array. The α-GPC pretreatment was identified to decrease the phosphorylation of heat shock protein 60 (HSP60), signal transducer and activator of transcription3 (STAT3), 90 kDa ribosomal S6 kinase 1/2 (RSK1/2), and p53, while increasing the phosphorylation of epidermal growth factor receptor (EGFR). B : One-way hierarchical clustering heatmap that represents non-treated controls and various treated groups. The heatmap was created using the Z-Score of normalized values from 1321 genes that exhibited a fold change greater than 2 and a statistical significance of p<0.05. C : Count of up- and down-regulated genes exhibiting a fold change greater than 2 and statistical significance of p<0.05, according to group comparisons. The number of up- or down-regulated genes increased depending on the administration of 2.5% isoflurane, compared to the variation in gene expression with the administration of 10 μM of α-GPC. D : Gene-set enrichment analysis using the Kyoto Encyclopedia of Genes and Genomes database. The comparative analysis between the ‘isoflurane 2.5%’ group and the ‘α-GPC 10.0 μM + isoflurane 2.5%’ group revealed distinct patterns. The most notable finding was the further increase in the gene ratio for ‘metabolic pathways’, which exhibited the highest gene ratio with statistical significance. α-GPC : L-α-Glycerophosphorylcholine, ECM : extracellular matrix.
Moreover, to investigate additional differentially expressed genes or pathways, we conducted transcriptome sequencing on a total of four groups. These groups included the control group and those treated with 10 µM of α-GPC and 2.5% isoflurane, either alone or in combination. A total of 1,321 genes satisfied a fold-change of more than two times and statistical significance of p<0.05 among the analyzed genes. Using the Z-score of normalized values, we performed one-way hierarchical clustering. As can be shown in the heatmap in Fig. 3B, differences in gene expression were noted among gene clusters in each group. The count of up- or down-regulated genes was higher depending upon whether 2.5% isoflurane was administered, compared to the change in gene expression depending on whether 10 µM of α-GPC was administered, among the 1321 verified genes in this study's setting (Fig. 3C). Additionally, a gene-set enrichment analysis was performed using the available gene information based on the KEGG database (Fig. 3D). The analysis revealed that 10 µM of α-GPC exhibited significant differences in the KEGG pathways related to breast cancer (gene ratio, 0.16; p<0.01) and regulation of the actin cytoskeleton (gene ratio, 0.16; p<0.05), compared to the ‘control’ group. In the group treated only with 2.5% isoflurane, notable changes were observed in KEGG pathways such as alcoholism (gene ratio, 0.11; p<0.001), neutrophil extracellular trap formation (gene ratio, 0.13; p<0.001), systemic lupus erythematosus (gene ratio, 0.11; p<0.001), pathways in cancer (gene ratio, 0.11; p<0.001), and metabolic pathways (gene ratio, 0.23; p<0.01), relative to the ‘control’ group. The comparison results between the ‘isoflurane 2.5%’ group and the ‘α-GPC 10.0 µM + isoflurane 2.5%’ group exhibited distinct patterns. The gene ratio of the metabolic pathways (gene ratio, 0.34; p<0.001) in the KEGG pathway increased further, and significant differences were observed in KEGG pathways such as human papillomavirus infection (gene ratio, 0.16; p<0.01), focal adhesion (gene ratio, 0.13; p<0.01), and ECM-receptor interaction (gene ratio, 0.09; p<0.01).
DISCUSSION
In this study, we demonstrated that pre-treatment with α-GPC had a detrimental effect on primary human astrocytes through the exacerbation of cytotoxicity under isoflurane-induced toxic conditions, which is in contrast with the well-known neuroprotective effect of α-GPC. These negative effects are believed to be due to decreased expression of antioxidant factors, such as HO-1 and HIF-1α, that protect astrocytes from oxidative stress, as suggested by the experimental results of this study. Although the interaction between α-GPC and isoflurane was unpredictable due to the absence of previous studies, we expected that α-GPC might exert a neuroprotective effect against isoflurane-induced toxicity, similar to its protective effect against ischemic damage. Contrary to this expectation, we found that pre-treatment with 10.0 µM of α-GPC further inflicted injury on primary human astrocytes exposed to 2.5% isoflurane.
Several recent studies have raised concerns that the efficacy and safety of α-GPC may not always be guaranteed depending on specific conditions, including tissue type, exposure duration, and dose. Tuboly et al. [37] reported conflicting results regarding α-GPC effects on cardiac cells. Short-term pre-exposure to α-GPC protected myocytes from ischemic damage by maintaining the physiological balance of reactive oxygen species production [37]. Conversely, long-term treatment with α-GPC was cytotoxic to cardiac cells by increasing oxidative stress levels and causing substantial cell death. Another recent population-based cohort study found that α-GPC users had a higher risk of total stroke, ischemic stroke, and hemorrhagic stroke compared to non-users [19]. This emphasizes that the appropriate use, dosage, and prescription duration of α-GPC are crucial. Considered together, our results suggest that the dose-dependent efficacy of α-GPC may have heterogeneous effects on cells, and draw attention to the safe therapeutic window of α-GPC.
Over the past several decades, substantial evidence has accumulated on the potential neurotoxic effects of isoflurane on neuronal survival, proliferation, and synaptogenesis. However, data on astrocytes are lacking and limited to animal models. There are few, but in some studies on isoflurane effects in astrocytes, isoflurane delayed astrocyte morphological differentiation and growth to a limited extent [9,25]. In contrast, other studies showed that apoptosis of astrocytes was precisely observed under overdose and prolonged exposure to isoflurane [14,41]. Therefore, the first step in this study was to determine the potential dose at which isoflurane causes cytotoxicity in human astrocytes. The results showed that cytotoxicity occurred in primary human astrocytes at concentrations greater than 2.5%. We established our experimental conditions for inducing isoflurane toxicity in vitro by administering 24 hours of isoflurane exposure, ensuring substantial toxicity induction. Twenty-four hours exposure to 2.5% isoflurane led to approximately a 14% decrease in cell viability (Fig. 2A, right), a relatively minor value, thereby eliminating the need for shorter isoflurane exposure. Even though the in vitro conditions allowed for longer treatment durations, 24 hours exposure to isoflurane significantly deviated from the standard duration observed in clinical settings. Collectively, we established that a singular 24 hours exposure to isoflurane was adequate to achieve our research objectives and attain the requisite experimental results, eliminating the need to extend the experiment beyond this timeframe. The 2.5% isoflurane is approximately equivalent to about 2.08 minimum alveolar concentration, which is close to 1.7–2.0 minimum alveolar concentration that blocks the adrenergic response to the incision in 50% of patients [24]. This concentration is slightly higher than the clinically applied dose, and 24 hours exposure is also relatively rare in clinics. However, a significant concentration of isoflurane was needed to induce cytotoxicity to set up the study design examining the effect of α-GPC pretreatment. Therefore, we considered this concentration acceptable for the experimental setup, along with the experimental results.
The decreased expression of survivin was consistent with decreased cell viability in the 10.0 µM of α-GPC pretreatment group exposed to 2.5% isoflurane. Although no statistically significant difference was observed between the ‘isoflurane 2.5%’ group and the ‘α-GPC 10 µM + isoflurane 2.5%’ group, both showed more than an 80% decrease in survivin expression. The noteworthy result here was the significant decrease in survivin expression observed in the group treated only with 10 µM of α-GPC. This suggests that while 10 µM α-GPC does not directly alter the cell viability of primary human astrocytes, it can induce a decrease in apoptosis inhibition, which could potentially enhance the vulnerability of the astrocytes to oxidative stress, one of the main mechanisms by which inhalation anesthesia induces toxicity [21,39]. In the case of HO-1, a previous study demonstrated that the factitious induction of HO-1 protected astrocytes from reactive oxygen species-induced oxidative stress, and this effect was hampered by HO-1 inhibitors, rendering astrocytes more susceptible to oxidative stress [15]. Consistent with the report, we observed a decrease in the viability of primary human astrocytes with decreased HO-1 expression by α-GPC pretreatment and exposure to the isoflurane-induced oxidative stress environment. The results indicate that pretreatment with 10 µM of α-GPC may increase vulnerability to oxidative stress. Another signal we have investigated, implicated in attenuating oxidative stress-induced apoptosis, is HIF-1α. This signal reportedly contributes to isoflurane-induced cytotoxicity. Accumulation of HIF-1α induced by isoflurane is known to act as a pro-apoptotic factor that results in cytotoxicity by releasing Ca2+ from the endoplasmic reticulum into the cytosol [6]. We observed that cell viability was lower in α-GPC pretreated astrocytes with a further reduction of HIF-1α than in the non-treated group which indicates reduction of HIF-1α expression by α-GPC pretreatment may have another crucial role in the enhanced isoflurane-induced toxicity.
The screening analysis of phosphorylation, which was additionally conducted to explore other related signals, revealed that several intracellular signaling factors related to oxidative stress-induced apoptosis were involved in α-GPC pretreatment. The decreased phosphorylation of HSP60, STAT3, RSK1/2, and p53 and increased phosphorylation of EGFR with the α-GPC pretreatment were identified. HSP60 and STAT3, which showed the most significant decrease in phosphorylation, are known to play a vital role in inducing apoptosis under severe oxidative stress conditions and in inhibiting production of superoxide anions and other reactive oxygen species, respectively [26,33]. RSK1 is recognized for reducing the generation of nitric oxide, a crucial molecule in oxidative stress [36]. The tumor suppressor, p53, is known to engage in p53-dependent transcription and the induction of apoptosis under oxidative stress conditions [35]. The EGFR, which significantly contributes to cell survival and growth, has been reported to activate signaling pathways under oxidative stress conditions [40].
In the KEGG pathway gene-set enrichment analysis, the results suggest that the alteration in the metabolic pathways of KEGG pathway observed between the ‘α-GPC 10 µM’ group and the ‘α-GPC 10.0 µM + isoflurane 2.5%’ group may be attributed to 2.5% isoflurane. In addition, while significant differences were observed in pathways in comparison results between the ‘isoflurane 2.5%’ group and the ‘α-GPC 10.0 µM + isoflurane 2.5%’, the most notable result was that the gene ratio for metabolic pathways increased further. Among the cell signals investigated through in vitro experiments, signals corresponding to the KEGG pathway results were HO-1, p53, and EGFR. HO-1 was identified as a cellular signal associated with metabolic pathways and p53 and EGFR were identified as associated with human papillomavirus infection in the KEGG database. Based on the cell viability assay results, in conjunction with the gene ratio and p-value from the KEGG pathway, we postulate that the findings for p53 and EGFR likely represent compensatory signal alterations associated with increased susceptibility to oxidative stress. Furthermore, we propose that the metabolic pathways within the KEGG pathway, along with the associated HO-1, may play a more significant role in exacerbating the toxicity induced by isoflurane in the presence of α-GPC.
This study has limitations. First, this study was conducted in a specific environment that induces isoflurane-induced toxicity in vitro and does not reflect the situations that occur in general clinical practice. Since this study is regarded as pilot research that can pose a minor question about the relationship between GPC and isoflurane, more systematic and specific experiments should follow for its verification. Secondly, this was an in vitro study and there is an inherent limitation to translating this finding into clinical settings without subsequent in vivo experiments. To further verify the results of this study, in vivo studies using appropriate animal models and experimental plans must be conducted, as well as more detailed in vitro follow-up experiments. Thirdly, we explored the effect of α-GPC on primary human astrocytes, but astrocytes coexist with neurons in the brain. Therefore, further studies of astrocytes co-cultured with neurons will provide additional information on the impact of α-GPC pre-treatment on the interaction between neurons and astrocytes. However, the significance of this study lies in the fact that it is the first to investigate the potential impact of α-GPC on primary human astrocytes in the context of isoflurane-induced toxicity.
CONCLUSION
We demonstrated for the first time that pretreatment with 10.0 µM of α-GPC made primary human astrocytes more susceptible to isoflurane-induced toxicity than non-treated cells. Additionally, we found that this vulnerability was attributed to the reduced expression of antioxidant factors by α-GPC pretreatment. Thus, despite several limitations of our in vitro study, we suggest that these findings be considered in future investigations and that further studies extend our results in vivo to examine the dose-response relationship between α-GPC pre-treatment and isoflurane-induced toxicity, with a particular emphasis on potential side effects. In addition, we hope that this study will serve as an opportunity to initiate further research to better understand the molecular mechanisms underlying the effects of α-GPC, which is widely used for neuroprotection, on other diseases or related clinical situations.
Notes
Conflicts of interest
No potential conflict of interest relevant to this article was reported.
Informed consent
This type of study does not require informed consent.
Author contributions
Conceptualization : YSL, YJK, KC; Data curation : HJL, HRC, MB, YSL, KC; Formal analysis : HJL, YSL, MB, KC; Funding acquisition : KC; Methodology : HRC, YSL, KC; Project administration : YJK, KC; Visualization : HJL, YSL, KC; Writing - original draft : HJL, YSL, KC; Writing - review & editing : MB, YJK, KC
Data sharing
None
Preprint
None
Acknowledgements
We would like to thank Dae Hye Shin and Hae Young Ko for their sincere support and assistance. We would also like to thank Editage (www.editage.co.kr) for English language editing. This work was supported by funding from the National Research Foundation of the Republic of Korea (NRF-2020R1C1C1010686 and NRF-2019M3A9E2061791).